The antibacterial effect of penicillin was discovered by Alexander Fleming in 1929. He noted that a fungal colony had grown as a contaminant on an agar plate streaked with the bacterium Staphylococcus aureus, and that the bacterial colonies around the fungus were transparent, because their cells were lysing. Fleming had devoted much of his career to finding methods for treating wound infections, and immediately recognised the importance of a fungal metabolite that might be used to control bacteria. The substance was named penicillin, because the fungal contaminant was identified as Penicillium notatum. Fleming found that it was effective against many Gram positive bacteria in laboratory conditions, and he even used locally applied, crude preparations of this substance, from culture filtrates, to control eye infections. However, he could not purify this compound because of its instability, and it was not until the period of the Second World War (1939-1945) that two other British scientists, Florey and Chain, working in the USA, managed to produce the antibiotic on an industrial scale for widespread use. All three scientists shared the Nobel Prize for this work, and rightly so – penicillin rapidly became the “wonder drug” which saved literally millions of lives. It is still a “front line” antibiotic, in common use for some bacterial infections although the development of penicillin-resistance in several pathogenic bacteria now limits its effectiveness (see later).The action of penicillin is seen in Figure A. This shows an ‘overlay plate’, in which a central colony of the fungus Penicillium notatum was allowed to grow on agar for 5-6 days, then the plate was overlaid with a thin film of molten agar containing cells of the yellow bacterium, Micrococcus luteus. The production of penicillin by the fungus has created a zone of growth inhibition of the bacterium. This demonstration parallels what Alexander Fleming would have observed originally, although he saw inhibition and cellular lysis of the bacterium Staphylococcus aureus.Figure B shows the typical asexual sporing structures of a species of Penicillium. The spores are produced in chains from flask-shaped cells (phialides) which are found at the tips of a brush-like aerial structure. |
![]() |
Penicillin has an interesting mode of action: it prevents the cross-linking of small peptide chains in peptidoglycan, the main wall polymer of bacteria. Pre-existing cells are unaffected, but all newly produced cells grow abnormally, unable to maintain their wall rigidity, and they are susceptible to osmotic lysis.This morphogenetic effect of penicillin can be demonstrated by growing either Gram-positive or Gram-negative bacteria in the presence of sub-lethal concentrations of penicillin. The images below show Gram-stained cells of Bacillus cereus that had been cultured in the absence of penicillin (left-hand image) or in the presence of a low concentration of the penicillin derivative termed Ampicillin (right-hand image). By affecting the cross-linking of the bacterial cell wall, penicillin has caused the bacterium to grow as larger cells with less frequent cell divisions.![]() |
As shown in the diagram below, penicillin is not a single compound but a group of closely related compounds, all with the same basic ring-like structure (a beta-lactam) derived from two amino acids (valine and cysteine) via a tripeptide intermediate. The third amino acid of this tripeptide is replaced by an acyl group (R in the diagram below), and the nature of this acyl group confers specific properties on different types of penicillin. ![]() |
The two natural penicillins obtained from culture filtrates of Penicillium notatum or the closely related species P. chrysogenum are penicillin G (shown in the diagram) and the more acid-resistant penicillin V. They are active only against Gram-positive bacteria (which have a thick layer of peptidoglycan in the wall) and not against Gram-negative species, including many serious pathogens like Mycobacterium tuberculosis (the cause of tuberculosis). (see Gram reaction for further details). Nevertheless, the natural penicillins were extremely valuable for treating wound pathogens such as Staphylococcus in wartime Europe.An expanded role for the penicillins came from the discovery that natural penicillins can be modified chemically by removing the acyl group to leave 6-aminopenicillanic acid (see diagram above) and then adding acyl groups that confer new properties. These modern semi-synthetic penicillins such as Ampicillin, Carbenicillin (see diagram) and Oxacillin have various specific properties such as:resistance to stomach acids so that they can be taken orally,a degree of resistance to penicillinase (a penicillin-destroying enzyme produced by some bacteria)extended range of activity against some Gram-negative bacteria.Although the penicillins are still used clinically, their value has been diminished by the widespread development of resistance among target microorganisms and also by some people’s allergic reaction to penicillin. |
Other antibioticsThe phenomenal success of penicillin led to the search for other antibiotic-producing microorganisms, especially from soil environments. One of the early successes (1943) was the discovery of streptomycin from a soil actinomycete, Streptomyces griseus. As shown in Figures C and D, actinomycetes are bacteria that produce branching filaments rather like fungal hyphae, but only about 1 micrometre diameter. They also produce large numbers of dry, powdery spores from their aerial hyphae. |
![]() Figure C. Edge of an agar colony of the actinomycete, Streptomyces griseus, viewed at low magnification (x10 objective of a compound microscope). Like all actinomycetes, this species grows as narrow filaments, with aerial branches that end in chains of spores. The spirally shaped aerial spore chains typical of the genus Streptomyces are seen in this image. Fig. D. Higher magnification of some of the aerial hyphae and spore chains. |
Actinomycetes, especially Streptomyces species, have yielded most of the antibiotics used in clinical medicine today. Some examples are shown in the table below. Much useful information on these organisms can be found in Actinomycete-related WWW Sites (not on this server). Other bacteria, including Bacillus species (see Figure E), have yielded few useful antibiotics. Fungi also have yielded few useful antibiotics. Apart from penicillin, the most important antibiotics from fungi are the cephalosporins (beta-lactams with similar mode of action to penicillin, but with less allergenicity) and griseofulvin (from Penicillium griseofulvum and related species) which is used to treat althlete’s foot and related fungal infections of the skin. |
![]() |
Some clinically important antibioticsAntibioticProducer organismActivitySite or mode of actionPenicillinPenicillium chrysogenumGram-positive bacteriaWall synthesisCephalosporinCephalosporium acremoniumBroad spectrumWall synthesisGriseofulvinPenicillium griseofulvumDermatophytic fungiMicrotubulesBacitracinBacillus subtilisGram-positive bacteriaWall synthesisPolymyxin BBacillus polymyxaGram-negative bacteriaCell membraneAmphotericin BStreptomyces nodosusFungiCell membraneErythromycinStreptomyces erythreusGram-positive bacteriaProtein synthesisNeomycinStreptomyces fradiaeBroad spectrumProtein synthesisStreptomycinStreptomyces griseusGram-negative bacteriaProtein synthesisTetracyclineStreptomyces rimosusBroad spectrumProtein synthesisVancomycinStreptomyces orientalisGram-positive bacteriaProtein synthesisGentamicinMicromonospora purpureaBroad spectrumProtein synthesisRifamycinStreptomyces mediterraneiTuberculosisProtein synthesis |
Why are there so few clinically useful antibiotics?Several hundreds of compounds with antibiotic activity have been isolated from microorganisms over the years, but only a few of them are clinically useful. The reason for this is that only compounds with selective toxicity can be used clinically – they must be highly effective against a microorganism but have minimal toxicity to humans. In practice, this is expressed in terms of the therapeutic index – the ratio of the toxic dose to the therapeutic dose. The larger the index, the better is its therapeutic value.It will be seen from the table above, that most of the antibacterial agents act on bacterial wall synthesis or protein synthesis. Peptidoglycan is one of the major wall targets because it is found only in bacteria. Some of the other compounds target bacterial protein synthesis, because bacterial ribosomes (termed 70S ribosomes) are different from the ribosomes (80S) of humans and other eukaryotic organisms. Similarly, the one antifungal agent shown in the table (griseofulvin) binds specifically to the tubulin proteins that make up the microtubules of fungal cells; these tubulins are somewhat different from the tubulins of humans. |
Comparing the antibiotic sensitivity of different bacteria![]() Figure F. Antibiotic-sensitivity testing. Petri dishes were spread-inoculated with Staphylococcus albus (white growth) or Micrococcus luteus (yellow growth) before antibiotic assay “rings” were placed on the agar surface. The coloured disks at the end of each spoke of the rungs are impregnated with different antibiotics. Clockwise from the top (arrow) these are: Novobiocin, Penicillin G, Streptomycin (white disk), Tetracycline, Chloramphenicol, Erythromycin, Fusidic acid (green disk) and Methicillin. Clear zones of suppression of bacterial growth around the individual antibiotic disks are evidence of sensitivity to these antibiotics.The diameter of the clear zone is related to the initial antibiotic concentration (which differs for the antibiotics on the ring), its solubility and its diffusion rate through agar. Standard tests performed on many bacteria by the manufacturers of these assay disks enable the diameter of the clearing zone to be related to the minimum inhibitory concentration (MIC) of each antibiotic for the strain being tested. The MIC can then be compared with the known tissue levels of these antibiotics when they are administered to patients, to assess whether the antibiotics would be effective for treatment of particular pathogens. |
The emergence of antibiotic resistanceThe repeated or continued use of antibiotics creates selection pressure favouring the growth of antibiotic-resistant mutants. These can be detected by comparing the size of clearing zones (or even the complete absence of clearing zones) of bacterial strains in plate assays such as those above. By the use of these disks it is also possible to detect the occurrence of individual mutant cells with antibiotic resistance in a culture of a strain that is sensitive to antibiotics. An example of this is shown in Figure G (below). ![]() (1996 survey)Human (1996 or 1997/98)Cephalosporins (beta lactams, including penicillins)121,603314,498Tetracyclines323,15147,500Aminoglycosides (e.g. streptomycin)37,0585,409Macrolides (e.g. erythromycin)71,22247,696Selected data from: J. Harvey and L Mason. The Use and Misuse of Antibiotics in Agriculture. Part 1. Current Usage. Published December 1998 by Soil Association, Bristol, UK (email: info@soilassociation.org). Not all of the antibiotics listed in the publication are shown here.Antibiotic resistance in genetically modified cropsA further source of concern is the widespread use of antibiotic-resistance genes as “markers” in genetically modified crops. Most of the companies insert antibiotic-resistance genes as “markers” during the early stages of developing their GM crops. This enables the scientists to detect when the genes that they are most interested in (herbicide-resistant genes or insecticidal toxin genes, etc.) have been inserted into the crop. The antibiotic-resistance genes then have no further role to play, but they are not removed from the final product. This practice has met with criticism because of the potential that the antibiotic-resistance genes could be acquired by microorganisms. In some cases these marker genes confer resistance to “front-line” antibiotics such as the beta-lactams. |
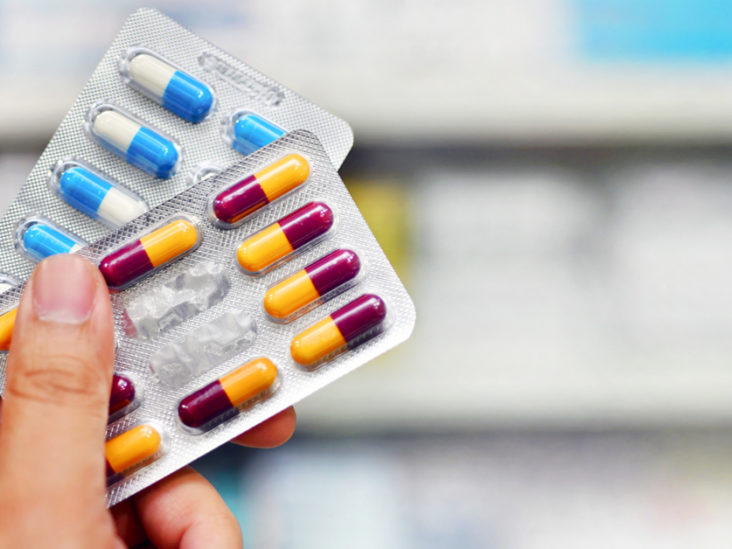
Posted inIndustrial Biotechnology Updates
Comments are closed